This article is part of the mini-monograph "World Trade Center Fine Particulate Matter--Chemistry and Toxic Respiratory Effects."
Address correspondence to S.H. Gavett, Pulmonary Toxicology Branch (Mail Code B143-02), U.S. EPA, Research Triangle Park, NC 27711 USA. Telephone: (919) 541-2555. Fax: (919) 541-0026. E-mail: gavett.stephen@epa.gov
Acknowledgments of personnel and financial support have been placed at the end of this article.
This paper has been reviewed and approved for release by NHEERL, U.S. EPA. Approval does not signify that the contents necessarily reflect the views and policies of the U.S. EPA, nor does mention of trade names or commercial products constitute endorsement or recommendation for use.
The authors declare they have no conflict of interest.
Received 12 August 2002; accepted 18 November 2002.
The attack on the World Trade Center (WTC) in New York City on 11 September 2001, in addition to causing the immediate and tragic loss of thousands of lives, resulted in the release of significant quantities of pollutants into the environment of the surrounding neighborhoods, including particulate matter (PM), asbestos, metals, and organic compounds [U.S. Environmental Protection Agency (U.S. EPA) 2002b]. Dust infiltrated indoors into homes and apartments, in many cases up to several inches in depth. Fires at the WTC site continued for several months before finally being extinguished, and recovery and reconstruction efforts contributed to emissions of fine [particulate matter with a mass median aerodynamic diameter (MMAD) < 2.5 µm; PM
2.5], coarse (> 2.5 and < 10 µm; PM
2.5-10), and larger (> 10 µm) PM fractions. As people have returned to their residences and businesses, potential exposure issues are associated with redispersal of residual dust.
Recent studies have assessed the chemical and physical properties of bulk samples of WTC PM. The dust particles from the WTC site appear to be quite alkaline in nature, probably because of partial dissolution of concrete, gypsum, and glass fiber particles [U.S. Geological Survey (USGS) 2002]. Lioy and co-workers (2002) found that bulk settled-dust samples of WTC PM were composed of particles of cement, carbon, cellulose, and several fiber types including mineral wool, fiberglass, cellulose, and asbestos. These authors also found significant concentrations of phthalate esters, polycyclic aromatic hydrocarbons (PAHs), and other hydrocarbons (Offenberg et al. 2003). However, a detailed analysis of the PM2.5 fraction of WTC dust has not been previously reported.
Exposure to ambient air PM has been associated with both acute and chronic adverse health effects, especially in sensitive populations (Dockery et al. 1993). Both fine- and coarse particles are readily inhaled into the human respiratory tract. Reviews of current literature suggest that fine particles are more strongly implicated in cardiovascular effects than coarse particles, while both impact respiratory end points (U.S. EPA 2002c). Fine particles are typically formed from gases or from combustion processes, whereas coarse particles are produced by mechanical breakdown of crustal material. However, the PM2.5 size range may also include a significant fraction of coarse particles that are broken down into smaller particles by strong mechanical forces (U.S. EPA 2002d). The unique situation of the WTC disaster may have generated a significant amount of crustal (building) material-derived particles in the PM2.5 size range. Although PM2.5-10 may have adverse health effects, quantities of WTC PM samples in this size range were limited (see "Size fractionation of bulk samples" in "Materials and Methods"). In addition, PM2.5 is very relevant toxicologically, as it is associated with epidemiologic findings of adverse health effects in humans (Dockery et al. 1993). Consequently, our objective in this article was to describe the collection, size fractionation, and chemical analysis of samples of WTC PM2.5 for use in toxicologic studies. To determine whether respirable WTC PM2.5 is more or less toxic than typical ambient air PM2.5, we compared the toxicity of WTC PM2.5 with well-characterized reference PM2.5 samples in a companion article (Gavett et al. 2003).
In this study we collected samples of settled dust from several sites in the immediate vicinity (< 0.5 miles) of Ground Zero on 12 and 13 September 2001. Samples were separated into fine, coarse, and larger fractions that could be studied for toxicologic effects. We thoroughly characterized the WTC PM2.5 samples using a number of chemical and physical techniques to compare the composition of these samples with other well-studied PM2.5 reference samples. Solid samples of WTC and reference PM2.5 were analyzed by scanning electron microscopy (SEM), X-ray diffraction (XRD), X-ray fluorescence (XRF), carbon fraction analysis, and neutron activation analysis (NAA). Analyses were also carried out on water-extracted or 1 M hydrochloric acid-extracted suspensions of WTC and reference PM2.5, including pH, endotoxin, and inductively coupled plasma-mass spectrometry (ICP-MS) and inductively coupled plasma-atomic emission spectrometry (ICP-AES). The analytical results will contribute to the understanding of the composition and possible mechanisms of toxicity of PM2.5 derived from the destruction of the WTC.
Detailed experimental findings of these studies are available in a U.S. EPA report (2002a).
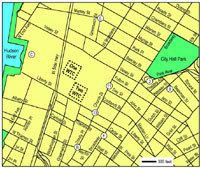 |
Figure 1. WTC dust samples were collected by New York University scientists from 13 sites on 12 September 2001 (numbers) and from 6 sites on 13 September 2001 (letters). Collection sites are shown only for samples reported in this article. Map reference: U.S. Census Bureau (2002).
|
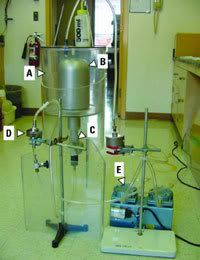 |
Figure 2. Apparatus used to separate bulk samples of WTC PM into different size fractions. Sieved WTC dust (PM53) was aerosolized in the resuspension chamber (A), then passed through a 10-µm cut inlet (B) and a 2.5-µm cut cyclone (C). PM2.5 were collected on Teflon filters (D) and pulled through by a pump (E).
|
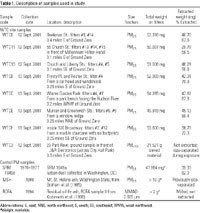 |
Table 1.
|
Collection of World Trade Center PM samples. Using a paper scoop, we sampled several outdoor locations as well as one indoor location, all of which appeared undisturbed since the collapse of the towers, as judged by the presence of a smooth uniform layer of dust and the absence of indicators of recent human activity. All samples were collected within a half-mile of Ground Zero and labeled with numbers (1-13) on 12 September 2001, and letters (A-F) on 13 September 2001. After examination of the available inventory of samples, we selected seven locations (8, 11, 13, B, C, E, and F) to assess toxicity of samples from different geographical locations as well as overall toxicity of a pooled sample from these locations (Figure 1). The locations were selected to represent a distribution surrounding Ground Zero, with more collection sites in the east reflecting the direction of the predominant winds during the collapse of the WTC towers and surrounding buildings.
Size fractionation of bulk samples. Bulk samples of the seven dust samples described above were sieved with a 53-µm diameter mesh screen (USA Standard Testing Sieves; Fisher Scientific, Pittsburgh, PA) on a shaker (Portable Sieve Shaker; Tyler Industrial Products, Mentor, OH). We processed the sieved material (PM53) in an apparatus designed to isolate different PM fractions (Figure 2). The PM53 fraction was aerosolized through a 10-µm aerodynamic diameter cut Wedding inlet (Anderson Instrument Co., Fultonville, NY) to remove particles in the 10-53 µm range and isolate the PM10 fraction. The PM10 fraction was then passed through a 2.5-µm cut cyclone (BGI, Inc., Waltham, MA) to remove the coarse fraction. The PM2.5 fraction was collected on Teflon filters (Zefluor Supported PTFE, 2-µm pore size, 47-mm diameter; Pall Gelman Sciences, Port Washington, NY). Ten to 40 filters were used in the size fractionation of each WTC sample. Roughly half the sample was in the PM53-sieved fraction. About 80-89% of the PM53 fraction was in the 10- to 53-µm size range, which is too large to use in respiratory toxicology studies, as deposition of particles > 5 µm is minimal in small laboratory animals (Raabe et al. 1988). The amount of the 2.5- to 10-µm fraction was very small (0.04-1.14% of the PM53 fraction, except 3.23% in sample 13) and was therefore not feasible to study. The PM2.5 fraction, however, was present in sufficient amounts (2.29-4.06% of PM53 fraction) to study for potential respiratory health effects. The sum of the size fraction percentages does not total 100% of the original PM53 fraction because of sample loss during fractionation. After overnight desiccation, filter weights were determined using an electrobalance (Model C-30; Thermo Cahn, Madison, WI). A total quantity of about 50 mg from each site, collected on 1-3 filters per site, was used in the study (Table 1). Mechanical scraping did not efficiently remove the PM2.5 from the filters, so it was necessary to isolate the PM2.5 using an aqueous extraction procedure.
Extraction of PM2.5 from Teflon filters. Filters containing the PM2.5 fraction were extracted using a modification of a method by Biran and co-workers (1996). Filters from each of the seven individual collection sites were extracted into distilled water (Gibco Invitrogen, Carlsbad, CA) in the ratio of 0.5 mL/mg sample (24.96-27.14 mL water). This volume of water was pipetted into a 100-mL sterile plastic cup containing a 3 mm-thick Teflon ring on the bottom designed to support the filter. Each filter was wetted with 200 µL 70% ethanol on the particle side, placed on top of the ring with the particle side down, and a 6 mm-thick Teflon ring was placed on top of the filter. The cup with the filter was placed on ice and secured to an orbital shaker (Titer Plate Shaker; Lab-Line Instruments, Melrose Park, IL). Samples were shaken at a low speed and sonicated for 30 min at a power setting of 30 (Sonic 300 Dismembrator; Artek Systems Corp., Farmingdale, NY). Ten milliliters was pipetted from each of the seven samples into a single sterilized 150-mL Erlenmeyer flask on ice to make a pooled sample (WTCX). The pH of the pooled and individual site samples was determined immediately after extraction (Model 440; Corning Incorporated, Corning, NY). Sonicated filters were desiccated before reweighing to determine quantity extracted (i.e., removed) from the filters (Table 1). Pooled and individual site samples were frozen at -80ƒC and lyophilized for 2 days at -55ƒC and 140 mtorr (Virtis Company, Gardiner, NY). Lyophilized samples were stored at 4ƒC and subsequently brought to room temperature for chemical or physical analysis, or for use in toxicologic studies in mice (Gavett et al. 2002).
Sample used for inhalation exposure toxicity testing. Because we needed to conduct a nose-only inhalation exposure study to assess upper airway responses of test animals (Gavett et al. 2003), a sufficient quantity of sample was necessary for the exposure system (> 2 g). As there was not enough PM2.5 or PM2.5-10 sample available to conduct this study, we decided to use a PM53 sample (sieved but not further fractionated) that was available in sufficient quantity to run through the inhalation exposure system. The inhalation exposure system uses a 2.5-µm cut cyclone to remove larger particles (Ledbetter et al. 1998), and therefore measurement of the PM concentration in the exposure zone of the chamber represents exposure to PM2.5. A sample of PM53 from location no. 3 (Figure 1; WTC3 in Table 1), 0.3 miles east of Ground Zero (in the predominant wind direction), was available in sufficient quantities for the nose-only inhalation exposure study.
Control PM samples. To assess the relative toxicity of WTC PM2.5, pooled and individual site samples were compared with three other well-characterized control PM2.5 samples. We chose samples representing an urban ambient PM with moderate toxicity, a crustal PM with low toxicity, and an emission source PM with high toxicity (Table 1). National Institute of Standards and Technology (NIST) Standard Reference Material (SRM) 1649a is an urban ambient PM collected in the Washington, DC, area in 1976-1977 over a 12-month period and represents a time-integrated sample (NIST 2001). This material was selected for comparison of toxicity of WTC PM2.5 with other typical urban air PM2.5 (albeit from an earlier era when leaded gasoline was still in use). This material was collected as a total suspended particulate (TSP) sample with a large amount of coarse PM and larger nonrespirable PM. Consequently, SRM 1649a was size-fractionated and extracted using the procedures described above for comparison with the WTC PM2.5 samples.
WTC PM2.5 was also compared with a previously size-separated PM2.5 fraction of ash from Mt. St. Helens (MSH) in Washington State (Graham et al. 1985). About half of MSH is crystalline in nature, primarily plagioclase, a series of compounds beginning with sodium aluminum silicate (NaAlSi3O8) and ending with calcium aluminum silicate (CaAl2Si2O8) that show continuous solid solution from albite to anorthite, with CaAl replacing NaSi as the series progresses. The remaining portion is amorphous (glass) and contains minor amounts of cristobalite (3%) and quartz (< 1%). The PM2.5 fraction of MSH has low toxicity in rats and mice (Hatch et al. 1984).
Residual oil fly ash (ROFA) is a PM sample with a high content of bioavailable transition metals including vanadium (V), nickel (Ni), and iron (Fe). Numerous studies have demonstrated that these metals are associated with lung injury in both healthy animals and animal models of cardiopulmonary injury (Dreher et al. 1997; Gavett et al. 1999; Kodavanti et al. 1998). For this study, we chose a sample of ROFA (no. 3; Kodavanti et al. 1998) from a boiler system that is toxic, yet not as soluble in water as previous samples of ROFA, and is therefore more comparable to WTC PM samples, which are not extremely water soluble. ROFA sample no. 3 has an MMAD of 2.665 µm, slightly larger than other PM2.5 samples used in this study but still acceptable for toxicity testing.
Overview of analytical methods. Analytical chemistry of WTC, SRM 1649a, MSH, and ROFA PM2.5 samples entailed measurements of both solid samples and their liquid extracts. Solid (including lyophilized) samples were characterized by SEM/energy dispersive X-ray analysis (SEM/EDX), XRD, NAA, XRF, and carbon fraction analysis of the organic, elemental, and carbonate fractions (OC/EC/CC). Deionized water extracts were characterized by pH, endotoxin, and ion chromatography (IC) analysis. Elemental and sulfate (SO4) content of deionized water and 1 M HCl extracts were measured using ICP-MS and ICP-AES. The two extraction liquids are used to estimate easily bioavailable and total bioavailable metal content, respectively. Although this speciation scheme is a rough approximation of bioavailability for PM2.5 samples of complex multielemental composition, it has proven useful in characterizing inhalation toxicology end points for various source and ambient particulates (Costa and Dreher 1997; Kodavanti et al. 1998).
Sufficient quantities of MSH, ROFA, and pooled WTCX samples were available to perform all analyses. Quantities of the individual site WTC samples and SRM 1649a were very limited (Table 1), and analyses were prioritized to yield the greatest information possible. SEM/EDX testing required < 1 mg and was selected for all samples. NAA was chosen as the next most applicable analysis to provide total elemental composition data to support SEM/EDX. We performed OC/EC/CC, ICP-MS, ICP-AES, and IC analyses on remaining samples where possible. XRD and XRF analyses were performed only on the WTC3 sample.
SEM/EDX analysis. SEM/EDX provided physical and chemical characterization of particles and fibers found in the control samples and lyophilized WTC PM2.5 samples, and on PM2.5 on polycarbonate filters taken during the inhalation exposure study using the WTC3 sample. SEM/EDX was carried out using a Personal SEM (PSEM) (formerly R.J. Lee Instruments, Ltd., now Aspex Instruments, Trafford, PA) as previously described (U.S. EPA 2002e). For lyophilized WTC or control samples, a small amount was applied to an adhesive carbon tab affixed to an aluminum SEM stub. For filter samples, small pieces (< 1 cm2) were affixed to aluminum SEM stubs using a carbonaceous suspension. Because only 15-30 images were examined from each sample, the results should not be interpreted as quantitative or comprehensive (Mamane et al. 2001). Rather, these qualitative results were used primarily to determine consistency with other analytical techniques described below.
X-Ray diffraction analysis. XRD was used to qualitatively determine the presence of any crystalline compounds in the WTC3 sample used in the inhalation exposure study. The PM53 sample was side-drifted into an aluminum holder and mounted into the Siemens D-500 diffractometer (Bruker Analytical X-Ray Systems, Madison, WI). The generator, set at 45 kilovolts and 40 milliamperes (mA), generated X-rays from a copper-target X-ray tube. The filament current was 3.46 mA. Intensities were collected by a lithium-drifted silicon detector fitted with a monochromator riding on a goniometer in the coupled
/2
mode, where
= diffraction angle. Peaks were collected in the range 2
= 5 to 85 degrees. Collection software used was Datascan (version 3.2; Materials Data, Inc., Livermore, CA). Evaluation software was MDI Jade 5 using the pattern library Powder Diffraction File (PDF), release 2000 (International Centre for Diffraction Data, Newtown Square, PA).
Neutron activation analysis. NAA was used to determine the total elemental content of lyophilized WTC and control PM2.5 samples (NCSU 2002). Samples of these materials (3-7 mg) were packaged in precleaned polyethylene vials in a clean hood, and irradiated as follows. For aluminum (Al), chlorine (Cl), copper (Cu), magnesium (Mg), manganese (Mn), sodium (Na), titanium (Ti), and V content, samples were irradiated for 30 sec at
900 kWth, decayed for 10-30 min, and counted for 5 min. For all other elements, samples were irradiated for 6 hr at
900 kWth. For arsenic (As), potassium (K), molybdenum (Mo), and antimony (Sb) content, samples were decayed for 1 week and counted for 15 min each. For Fe, Ni, barium (Ba), calcium (Ca), chromium (Cr), strontium (Sr), and zinc (Zn) content, samples were decayed for 2 weeks and counted for 30 min each.
-Ray spectra were counted and compared against calibration and quality control standards, and quantitative analyses were performed.
X-Ray fluorescence analysis. Five polycarbonate filters (Isopore 0.8 µm, 47-mm diameter, Millipore Corporation, Bedford, MA) loaded with the WTC3 PM2.5 sample, with five lot-matched blank filters, were mounted in liquid-type polyethylene sample cups, placed in a stainless steel sample holder, and analyzed. X-ray intensities were collected with the Philips PW2404 XRF (Philips Analytical, Natick, MA) using the Philips proprietary software SuperQ. Net intensities (peak-background) were analyzed using the standardless software UniQuant 4 (Omega Data Systems, Veldhoven, the Netherlands) after subtraction of the intensities due to the blank filter system. The constituents of the dust were evaluated as oxides.
Carbon fraction analysis. Carbon speciation into OC/EC/CC fractions was performed on control and lyophilized WTC PM2.5 samples and on quartz filters taken during the inhalation exposure to WTC3 PM2.5. The thermo-optical method, based upon sequential pyrolytic vaporization and detection of the three carbon fractions, was performed (Sunset Laboratory, Forest Grove, OR) as previously described (Birch and Cary 1996; Sunset 2002).
Endotoxin analysis. One-milliliter aliquots of filter extracts from each individual site sample were analyzed for endotoxin content using the limulus amebocyte lysate gel-clot method (Associates of Cape Cod, Inc., Falmouth, MA). Samples were titered using a 2-fold dilution scheme against control standard endotoxin [CSE; lot no. 85, Escherichia coli O113, 5 endotoxin units (EU)/ng]. Preliminary inhibition tests (positive product controls) were performed on the undiluted samples spiked with CSE equivalent to twice the sensitivity (
; 0.03 EU/mL). The error of the gel-clot method is ± one 2-fold dilution.
ICP-MS and ICP-AES. Control PM2.5 samples, lyophilized WTC PM2.5 samples, and polycarbonate filters taken during the inhalation exposure to WTC3 PM2.5 were extracted with deionized water or 1 M HCl and analyzed for their elemental content. Samples (4-6 mg) were extracted with 1.6 mL of either liquid. Polycarbonate filters containing 2-3 mg WTC3 PM2.5 were extracted with 13 mL of either liquid. High-speed centrifugation was used to separate the liquid and solids (17,000
g for 1.6-mL samples; 51,000
g for 13-mL samples). After dilution, extraction solutions were analyzed quantitatively using ICP-MS (ELAN 6000; PerkinElmer Instruments, Shelton, CT) and ICP-AES (Model P40; PerkinElmer Instruments) as previously described (U.S. EPA 2002f, 2002g, respectively). Filter blanks levels for all elements were negligible compared with the levels in the PM samples.
Ion chromatography of deionized water extracts. Deionized water extracts from the ICP sample prep as described above were analyzed quantitatively for anion and cation content using IC (ManTech Environmental, Research Triangle Park, NC). A DX-500 ion chromatograph (Dionex, Sunnyvale, CA) was used with an AS14 column (Dionex) for anion analysis in Suppressor-ASRS Ultra-AutoSuppressor Recycle mode, with 3.5 mM Na2CO3/1.0 mM NaHCO3 eluent. A CS12 column (Dionex) was used for cation analysis in Suppressor-CSRS II Ultra-AutoSuppressor Recycle mode, with 20 mM methanesulfonic acid eluent.
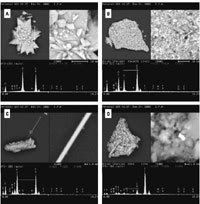 |
Figure 3. SEM/EDX results from water-extracted lyophilized WTCX PM sample. The upper-left quadrant of each photomicrograph shows a field of view with the particle of interest within the smaller square in that field. The upper-right quadrant shows a zoomed-in view of the feature (the area from within the square in the upper-left quadrant), and the lower half shows the elemental spectrum acquired with the electron beam centered on the small (barely visible) square in the zoomed-in view. (A) Example of crystal with Ca, S, and O, which dominated the samples. (B) Example of fine particle aggregate that was prominent in the samples. (C) Example of fiber found in the samples. (D) Example of metallic particle (Ti) within fine particle aggregate.
|
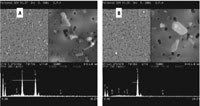 |
Figure 4. SEM/EDX results showing predominant particle types found in aerodynamically size-separated WTC3 PM2.5 sample used in nose-only inhalation exposure study (Gavett et al. 2003). (A) Example of particle containing Ca, S, O, and carbon. (B) Example of particle containing Ca, O, and carbon without significant levels of S.
|
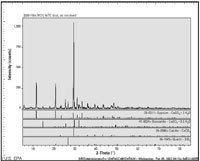 |
Figure 5. X-Ray diffraction analysis of sieved WTC3 sample (PM53 before aerodynamic size separation). Peaks were collected in the range of 2 = 5-85ƒ. Collection software used was Datascan (version 3.2; Materials Data, Inc.).
|
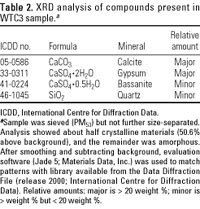 |
Table 2.
|
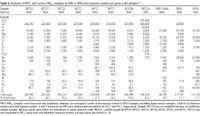 |
Table 3.
|
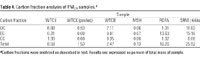 |
Table 4.
|
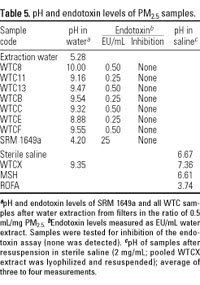 |
Table 5.
|
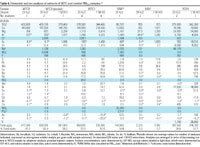 |
Table 6.
|
Analytical chemistry of solid samples. SEM/EDX analysis. Water-extracted and lyophilized WTC PM2.5 samples were dominated by large snowflakelike crystals composed of Ca, sulfur (S), and oxygen (O) (Figure 3A). Aggregates of fine particles composed of various combinations of Ca, Mg, Al, S, O, and silicon (Si) were also prominent (Figure 3B). Fibers approximately 1 µm in diameter were found in most of the samples and had a composition similar to the fine particle aggregates (Figure 3C). Metallic particles [mostly Ti and Fe, although Zn, lead (Pb), Ba, and Cu were also found] were found typically as inclusions in the large aggregates of fine particles (Figure 3D).
SEM/EDX analysis of the aerosolized PM2.5 fraction of WTC3 (not water extracted) showed the same overall chemistry as the extracted and lyophilized WTC PM samples; the majority of particles were composed of Ca, carbon, and O with S (Figure 4A) [or without significant levels of S (Figure 4B)] and some also contained Si. In contrast to the crystals and aggregates of the lyophilized samples described above and shown in Figure 3, the WTC3 particles were small, typically about 1 µm, with rough, irregular features. The different physical form of the Ca-based particles in WTC3 reflects the dry size segregation of the inhalation exposure system. These results suggest that the large crystals and aggregates observed in the lyophilized samples were not originally present in the PM2.5 Teflon filters but were formed during the aqueous extraction and lyophilization process.
Particles with other compositions were found with far less frequency. These included particles composed of Fe, carbon, and Sb-Zn (one example found). One or more possible asbestos fibers (Mg-Si composition) were also found; however, polarized light microscopy rather than SEM/EDX is the preferred method for identifying asbestos in bulk materials. SEM/EDX analysis performed on MSH, SRM 1649a PM2.5, and ROFA showed results typically found in previous analyses of these materials (data not shown).
X-Ray diffraction analysis. XRD analysis of WTC3 PM53 (before size segregation by the inhalation exposure system) showed a complex pattern containing 25 peaks, indicating the presence of several crystalline materials. The peak area above the background curve was 50.6%. The 49.4% below the curve indicated that WTC3 PM53 consisted of about half amorphous materials. Four patterns were identified as being consistent with peaks identified in the dust. Figure 5 shows the XRD spectra of WTC3 PM53 and those of the matched compounds. Two compounds were identified as major constituents [calcium sulfate dihydrate (gypsum) and calcium carbonate (calcite)], and two were identified as minor constituents [bassanite (calcium sulfate hemihydrate) and quartz (silicon dioxide); Table 2]. The predominance of Ca-based compounds by XRD supports the major findings of the SEM/EDX analysis of WTC PM2.5--the WTC PM materials are composed predominantly of Ca compounds, some of which are water soluble. The sample of MSH was also analyzed by XRD, and the results were consistent with those previously reported (Graham et al. 1985; data not shown).
Total elemental analysis by neutron activation analysis and X-ray fluorescence analysis. Both NAA and XRF analyses showed that there were large amounts (range 22-33%) of Ca in the WTC PM2.5 samples (Table 3). XRF analysis showed 37.5% S as SO4 in the WTC3 PM2.5 sample. Together, Ca and SO4 account for 64% of the WTC3 PM2.5 sample, in good agreement with the SEM/EDX and XRD analyses. For all other elements, NAA and XRF results for the various WTC PM2.5 samples show good agreement. This consistency in composition is remarkable, considering the wide area over which the original WTC dust samples were collected (Figure 1). Besides Ca and SO4, only Si, Al, Mg, and Fe are present in levels approaching or greater than 1% of total mass. The relative weight-percent ratios of Al, Mg, and Fe are in the range of those found in portland cement, a major component of concrete (McKetta 1978). Of the transition and heavy metals and other toxic elements such as Sb and As, only Zn and Ti are present in levels approaching 0.5% of total mass, whereas all others are present at maximum levels of hundreds of micrograms per gram (parts per million). Within the group of seven WTC PM2.5 samples analyzed using NAA, WTCE has the highest content of total metals and toxic elements (e.g., Sb and As); combined levels of these elements were about twice those of the other WTC samples. Comparison of the total metal and toxic elemental content of the WTC PM2.5 samples with the control dusts shows the overall metal levels as MSH < WTC < SRM 1649a < ROFA.
Carbon fraction analysis. The WTC PM2.5 samples had low total carbon content, in the range of 1.5-8.5% (Table 4), in contrast with control samples such as SRM 1649a (26%) and ROFA (16%). MSH had almost no carbon, as expected from this crustal PM sample. The WTC3 PM2.5 sample used in the inhalation study had about 4 times as much carbon as the other two WTC PM2.5 samples. This result may be due to differences in the method by which the samples were isolated (physical separation vs. aqueous extraction and lyophilization) or may simply be due to variability in carbon content of samples from different locations. Despite the variation in total carbon content of WTC PM samples, the ratios of the carbon fractions were similar. Carbonate carbon is most likely associated with Ca (as calcite, Table 2) and other alkaline earth metals (e.g., Mg). The level of carbonate carbon is relatively small compared with the overall alkaline earth content, yet levels are high enough to make Ca and/or other alkali earth carbonates the likely source(s) of the high pH values measured (see below). Elemental carbon levels (an indicator of carbon black produced by incomplete combustion during the fires prior to collapse) were low, indicating that the fine fraction of WTC PM2.5 was dominated by the presence of pulverized building materials rather than the products of incomplete combustion. Organic carbon levels are relatively high and can be attributed to many sources in the WTC building and to jet fuel (Lioy et al. 2002).
Analytical chemistry of aqueous extracts of samples. pH and endotoxin levels. pH levels of water-extracted WTC PM2.5 and control samples are shown in Table 5. The pH of water-extracted WTC PM2.5 samples before lyophilization ranged from 8.88 in WTCE to 10.00 in WTC8. The alkaline pH results from the building materials comprising much of the dust, most likely the alkaline earth (Ca, Mg) compounds. Calcium carbonate, identified by XRD, is a major component of cement (McKetta 1978) and other building materials. It is almost insoluble in water (14 µg/mL), yet a saturated solution produces a pH of 9.4 (Weast 1985). For a 2 mg PM/mL ratio, this requires < 7 µg soluble calcium carbonate/mg PM. Such a level is easily attainable in the WTC samples, given the percent ranges of Ca and carbonate carbon measured by elemental and OC/EC/CC analysis. The pH of lyophilized WTC PM2.5 reconstituted in unbuffered saline was very close to neutral (pH 7.36), whereas MSH was only slightly acidic (pH 6.61) and ROFA was moderately acidic (pH 3.74). It is not known why the pH of WTC PM2.5 should be close to neutral after reconstitution in saline.
Endotoxin levels in WTC PM2.5 samples were minimal compared with other urban PM samples such as SRM 1649a, which was also low (Table 5). Several thousand times this level of endotoxin caused an acute neutrophilic response in the lungs of CD-1 mice (Dhingra et al. 2001). The level of endotoxin in the samples used in this study would not be anticipated to contribute directly to any inflammatory response in the lungs.
Extractable elemental/ion analysis by ICP-MS, ICP-AES, and IC. Elemental and ion compositions were consistent within each sample group tested (Table 6). The ICP-AES data for S were treated as SO4, as both XRD and XRF showed S was present in this oxidized form. The SO4 content of the WTC PM2.5 samples ranged from 38 to 44% of total weight, and the WTC3 ICP-AES and XRF results (Table 3) were nearly identical. The ICP-AES data showed that water-soluble Ca accounted for 18-19% of WTC PM2.5 samples, which was about 8-15% lower than results from XRF and NAA analyses (Table 3). This may reflect an incomplete extraction in the 1-hr time frame, the relatively mild solutions and conditions used in the extraction method, or the presence of other insoluble forms of Ca in the WTC PM2.5 samples. ICP data for the 1 M HCl-soluble extracts of WTC PM2.5 showed an additional 1-2 weight percent Ca content relative to the water-soluble extracts. The increase may be attributed to calcite or other water-insoluble Ca salts that are soluble in 1 M HCl.
The same ordering of water-soluble element < 1 M HCl-soluble element < total element content held for all other analytes. Total content measured by NAA/XRF and acid-soluble content measured by ICP agreed within 25% for elements such as Mg and Zn, which exist in compounds more amenable to acid dissolution (Budavari 1996; Weast 1985). Elements such as Al, Fe, and Ti, which are in the form of complex oxides usually in combination with Si, are much less soluble under the acid extraction conditions used in this study and do not agree as well (Budavari 1996; Weast 1985). Water-extractive contents were < 10% of the total content values for nearly all transition and heavy metals. Acid-extractive contents were almost all < 50% of the total content values.
Comparison of the water-soluble transition and heavy metal levels of the WTC samples with the control dusts used in this study shows the overall metal content trend as MSH < WTC < SRM 1649a < ROFA. ROFA has high levels of water-soluble transition metals, including V, Ni, and Fe, which are important in its toxicity (Kodavanti et al. 1998). The 1 M HCl-soluble metal content trend is MSH < WTC < ROFA (not enough SRM 1649a PM2.5 sample was available to run this test). Extractable elemental content of the WTC samples more closely resembled the crustal MSH sample than the urban ambient SRM 1649a PM2.5 sample.
We collected samples of fallen dust at various locations in the immediate vicinity of the WTC site 1 and 2 days after the WTC disaster and analyzed the PM
2.5 fraction, using several physical and chemical techniques. The XRD analysis showed that the sieved WTC PM
53 sample consists primarily of Ca-based compounds, specifically calcium sulfate (gypsum) and calcium carbonate (calcite; the main component of limestone). The NAA, XRF, and ICP analyses strongly suggest that these compounds are present in the PM
2.5 fraction as well. Ca and SO
4 compose about two-thirds of WTC PM
2.5 on a weight percent basis. Taken together, the total elemental analysis data account for 79% of the weight of the WTC3 PM
2.5 sample and indicate that the main components are construction materials such as cement, concrete aggregate, ceiling tiles, and wallboard. The remaining mass fraction is most likely due to moisture content and to O, hydrogen, and nitrogen associated with both the organic and inorganic constituents.
The content of transition and heavy metals and other toxic trace elements in WTC PM2.5 samples is low compared with that of combustion-derived ROFA, as expected from crustal-derived building materials. Levels of elements that could be attributed to metal wiring (Cu), plumbing (Fe, Cu, Pb), structural steel (Fe, Mn), and communication and computer equipment (Cu, Fe, Zn, others) are also low. This may be attributed to the relatively small proportion of metal-containing building contents compared with the building itself, or perhaps these materials resisted crumbling and pulverization into the PM2.5 fraction. Elemental analysis of water and acid extracts of WTC PM2.5 provides speciation information on chemical constituents that are potentially bioavailable after inhalation, or which can be easily released into the environment from rain and washing of streets and buildings. The extractable amounts of metals and toxic elements are low compared with those of the ROFA and ambient air SRM 1649a samples, partly because of the small total amounts present in the original material, and partly because of the limited solubility of most of these elements at the alkaline pH levels (8.9-10.0) of the water extracts (Hoffland 2002; Neubauer et al. 2000).
The results of this study differ in some respects from those of the USGS (2002) and Lioy and co-workers (2002), who performed chemical and physical analyses of whole (not size-separated) WTC PM samples collected within a 3-km radius of the WTC site. The USGS (2002) collected several dust samples on 17 and 18 September that had only limited protection from the rain that fell on 14 September and possibly changed sample composition. The USGS study found Ca in the whole dust approximately in the same proportion (average 18%) that we found in the PM2.5 fraction, but S averaged 3% (or 9% as SO4), a level about 4-fold lower than reported here, suggesting that SO4 may have been washed away by rain more easily than other species. The USGS also found a notably higher level of Si (average 15% vs. 3% here), probably reflective of a large amount of glass fiber present in very coarse fractions (Lioy et al. 2002; USGS 2002), which do not fragment into PM2.5 as easily as gypsum and other building materials. Lioy and co-workers (2002) collected three samples on 16 and 17 September from sheltered locations and found that Ca and SO4 in the settled dust were present in the same ratio as in our study. However, the combined levels comprised about 6% of the whole dust, a level about 10-fold lower than that found in the PM2.5 fraction in our study.
We conclude that water-soluble Ca-containing compounds were enriched in the WTC PM2.5 fraction compared with those in the whole settled dust. Additionally, the WTC PM2.5 samples were remarkably homogeneous in their overall elemental content, considering the wide geographic range of sample collection. These results were unexpected, given the complexity of the building material composition and scale of the disaster. However, they are reasonable, considering the prevalent use of gypsum in building materials such as ceiling tiles, wallboard, and cement, and the ease with which these materials can be crumbled into a fine powder, mix, and homogenize.
The likely major acute inhalation hazards of WTC PM2.5 based on the results from this study are due to the presence of gypsum, calcite, and cement or concrete dust components. Both gypsum and calcite irritate the mucus membranes of the eyes, nose, throat, and upper airways (Stellman 1998). Calcium carbonate dust causes coughing, sneezing, and nasal irritation (NLM 2002). These symptoms of inhalation exposure are similar to those reported by rescue and cleanup workers in the immediate aftermath of the WTC attack (Kelley 2001). The high content of gypsum and calcite in the WTC PM2.5 fraction suggests that potentially toxic effects may also extend into the smaller airways and lung parenchyma.
The physical and chemical characterization of the samples described here contributes to the understanding of biologic responses in the companion article (Gavett et al. 2003), which describes short-term acute respiratory tract toxicology of the WTC PM2.5 and control PM2.5 samples. Further detailed analyses and toxicity testing of the WTC PM2.5 fraction may be necessary to evaluate other components found in the bulk WTC fallout dust, which may be enriched in the smaller inhalable particle sizes, and which have known long-term health consequences. Identified WTC bulk PM components that are known inhalation hazards are asbestos, crystalline silica, and toxic organics such as PAHs, polychlorinated biphenyls, and dioxins (Lioy et al. 2002; Offenberg et al. 2003; USGS 2002). Calcite and gypsum are often contaminated with small amounts of silica, which is the main concern for long-term occupational health hazards (Stellman 1998). Minor amounts of silica were detected in the WTC PM samples (Table 2). The results of this study are also of special interest because of resuspension of the dust during cleanup operations of both the Ground Zero site and building interior surfaces throughout lower Manhattan (Johnson 2002). WTC PM that infiltrated into buildings is most likely of smaller particle sizes and more of an inhalation hazard than that found outdoors.
Acknowledgments: The authors thank M. Zhong, M. Blaustein, S-I. Hsu, J. Duffy, K. Schermerhorn, M. Lippmann, J. Zelikoff, and M. Costa (New York University School of Medicine); J. Vandenberg, T. Hughes, B. Culpepper, I. Gilmour, J. Lehmann, and H. Hilliard (NHEERL, ORD, U.S. EPA); G. Ross (National Caucus for Black and Aging/Senior Environmental Employees Program, Research Triangle Park, NC); D. Williams and W. Ellenson (ManTech Environmental Technology, Inc., Research Triangle Park, NC); R. Cary and D. Smith (Sunset Laboratory, Forest Grove, OR); and S. Lassell (Department of Nuclear Engineering, North Carolina State University, Raleigh, NC) for their excellent assistance.
LCC and MDC were supported by National Institute of Environmental Health Sciences center grant ES00260 and U.S. EPA PM center grant R827351.
References
Biran R, Tang YZ, Brook JR, Vincent R, Keeler GJ. 1996. Aqueous extraction of airborne particulate matter collected on hi-vol Teflon filters. Int J Environ Anal Chem 63:315-322.
Birch ME, Cary RA. 1996. Elemental carbon-based method for monitoring occupational exposures to particulate diesel exhaust. Aerosol Sci Technol 25:221-241.
Budavari S, ed. 1996. The Merck Index. 12th ed. Whitehouse Station, NJ:Merck & Co., Inc.
Costa DL, Dreher KL. 1997. Bioavailable transition metals in particulate matter mediate cardiopulmonary injury in healthy and compromised animal models. Environ Health Perspect 105(suppl 5):1053-1060.
Dhingra VK, Uusaro A, Holmes CL, Walley KR. 2001. Attenuation of lung inflammation by adrenergic agonists in murine acute lung injury. Anesthesiology 95:947-953.
Dockery DW, Pope CA 3rd, Xu X, Spengler JD, Ware JH, Fay ME, et al. 1993. An association between air pollution and mortality in six U.S. cities. N Engl J Med 329:1753-1759.
Dreher KL, Jaskot RH, Lehmann JR, Richards JH, McGee JK, Ghio AJ, et al. 1997. Soluble transition metals mediate residual oil fly ash induced acute lung injury. J Toxicol Environ Health 50:285-305.
Gavett SH, Haykal-Coates N, Highfill JW, Ledbetter AD, Chen LC, Cohen MD, et al. 2003. World Trade Center fine particulate matter causes respiratory tract hyperresponsiveness in mice. Environ Health Perspect 111:981-991 (2003).
Gavett SH, Madison SL, Stevens MA, Costa DL. 1999. Residual oil fly ash amplifies allergic cytokines, airway responsiveness, and inflammation in mice. Am J Respir Crit Care Med 160:1897-1904.
Graham JA, Miller FJ, Davies DW, Hiteshew ME, Walsh LC III. 1985. Inhalation studies of Mt. St. Helens volcanic ash in animals. I. Introduction and exposure system. Environ Res 37:61-71.
Hatch GE, Raub JA, Graham JA. 1984. Functional and biochemical indicators of pneumoconiosis in mice: comparison with rats. J Toxicol Environ Health 13:487-497.
Hoffland. 2002. Hydroxide Precipitation of Metals. Conroe, TX:Hoffland Environmental, Inc. Available: http://www.hofflandenv.com/?structure=./contents/tks/3.xml [accessed 28 October 2002].
Johnson K. 2002. As 9/11 cleanup moves inside, residents battle with emotions. New York Times (New York, NY) 1 July:A1.
Kelley T. 2001. At least a quarter of Ground Zero firefighters ill. New York Times (New York, NY) 21 December:A1.
Kodavanti UP, Hauser R, Christiani DC, Meng ZH, McGee J, Ledbetter A, et al. 1998. Pulmonary responses to oil fly ash particles in the rat differ by virtue of their specific soluble metals. Toxicol Sci 43:204-212.
Ledbetter AD, Killough PM, Hudson GF. 1998. A low-sample-consumption dry-particulate aerosol generator for use in nose-only inhalation exposures. Inhal Toxicol 10:239-251.
Lioy PJ, Weisel CP, Millette JR, Eisenreich S, Vallero D, Offenberg J, et al. 2002. Characterization of the dust/smoke aerosol that settled east of the World Trade Center (WTC) in lower Manhattan after the collapse of the WTC 11 September 2001. Environ Health Perspect 110:703-714.
Mamane Y, Willis RD, Conner TL. 2001. Evaluation of computer-controlled scanning electron microscopy applied to an ambient urban aerosol sample. Aerosol Sci Technol 34:97-107.
McKetta JJ, ed. 1978. Cements, Portland. In: Encyclopedia of Chemical Processing and Design. New York:Marcel Dekker, 82-93.
NCSU (North Carolina State University). 2002. Nuclear Reactor Program: Neutron Activation Analysis. Available: http://www.ne.ncsu.edu/NRP/reactor_program.html [accessed 29 October 2002].
Neubauer U, Mowack B, Furrer G, Schulin R. 2000. Heavy metal sorption of clay minerals affected by the siderophore desferroxamine B. Environ Sci Technol 34:2749-2755.
NIST. 2001. Certificate of Analysis: Standard Reference Material 1649a. Gaithersburg, MD:National Institute of Standards and Technology, 1-22.
NLM (National Library of Medicine). 2002. Hazardous Substances Data Bank. Available: http://toxnet.nlm.nih.gov/ [accessed 28 October 2002].
Offenberg JH, Eisenreich SJ, Chen LC, Cohen MD, Chee G, Prophete C, et al. 2003. Persistent organic pollutants in the dusts that settled across lower Manhattan after September 11, 2001. Environ Sci Technol 37:502-508.
Raabe OG, Al-Bayati MA, Teague SV, Rasolt A. 1988. Regional deposition of inhaled monodisperse coarse and fine aerosol particles in small laboratory animals. In: Inhaled Particles VI (Dodgson J, McCallum RI, Bailey MR, Fisher DR, eds). Oxford, UK:Pergamon Press, 53-63.
Stellman, JM, ed. 1998. Encyclopaedia of Occupational Health and Safety, 4th ed. Geneva:International Labour Office.
Sunset. 2002. Sample Analysis Method for Organic and Elemental Carbon Aerosols. Forest Grove, OR:Sunset Laboratory, Inc. Available: http://www.sunlab.com/SampleAnalysisMethod.html [accessed 28 October 2002].
U.S. Census Bureau. 2002. Cartographic Products. On-Line Mapping Resources. TIGER Mapping Services. Washington, DC:U.S. Census Bureau. Available: http://tiger.census.gov/cgi-bin/mapbrowse-tbl [accessed 28 October 2002].
U.S. EPA. 2002a. Toxicological Effects of Fine Particulate Matter Derived from the Destruction of the World Trade Center. EPA/600/R-02/028. Cincinnati, OH:U.S. Environmental Protection Agency. Available: http://www.epa.gov/nheerl/wtc./WTC_report_7b3i.pdf [accessed 2 April 2003].
------. 2002b. EPA Response to September 11: Daily Environmental Monitoring Summary. Washington, DC:U.S. Environmental Protection Agency. Available: http://www.epa.gov/wtc/data_summary.htm [accessed 28 October 2002].
------. 2002c. Third External Review Draft of Air Quality Criteria for Particulate Matter (April 2002). Vol II. Research Triangle Park, NC:U.S. Environmental Protection Agency, 8-237. Available: http://www.epa.gov/ncea/pdfs/partmatt/VOL_I_AQCD_PM_3rd_Review_Draft.pdf [accessed 28 October 2002].
------. 2002d. Third External Review Draft of Air Quality Criteria for Particulate Matter (April 2002). Vol I. Research Triangle Park, NC:U.S. Environmental Protection Agency, 2-15. Available: http://www.epa.gov/ncea/pdfs/partmatt/ [accessed 28 October 2002].
------. 2002e. Guidelines for the Application of SEM/EDX Analytical Techniques to Particulate Matter Samples. EPA/600/R-02/070. Cincinnati, OH:U.S. Environmental Protection Agency.
------. 2002f. Method 6020a: Inductively Coupled Plasma-Mass Spectrometry. EPA SW-846. Washington, DC:U.S. Environmental Protection Agency. Available: http://www.epa.gov/epaoswer/hazwaste/test/pdfs/6020a.pdf [accessed 28 October 2002].
------. 2002g. Methods for the Determination of Metals in Environmental Samples. Method 200.7: Determination of Metals and Trace Elements by Inductively Coupled Plasma-Atomic Emission Spectrometry. EPA/600/4-91/010. Cincinnati, OH:U.S. Environmental Protection Agency. Available: enter"600491010" on search of: http://www.epa.gov/cgi-bin/claritgw?op-NewSearch&template=epa [accessed 28 October 2002].
USGS. 2002. Environmental Studies of the World Trade Center Area after the September 11, 2001 Attack. Open File Report OFR-01-0429. Denver, CO:U.S. Geological Survey. Available: http://geology.cr.usgs.gov/pub/open-file-reports/ofr-01-0429/ [accessed 28 October 2002].
Weast RC, ed. 1985. CRC Handbook of Chemistry and Physics. 66th ed. Boca Raton, FL:CRC Press, Inc.
Last Updated: May 19, 2002